October 1, 1994
17 min read

The Evolution of the Universe
Some 15 billion years ago the universe emerged from a hot, dense sea of matter and energy. As the cosmos expanded and cooled, it spawned galaxies, stars, planets and life
By P. James E. Peebles , David N. Schramm , Edwin L. Turner & Richard G. Kron
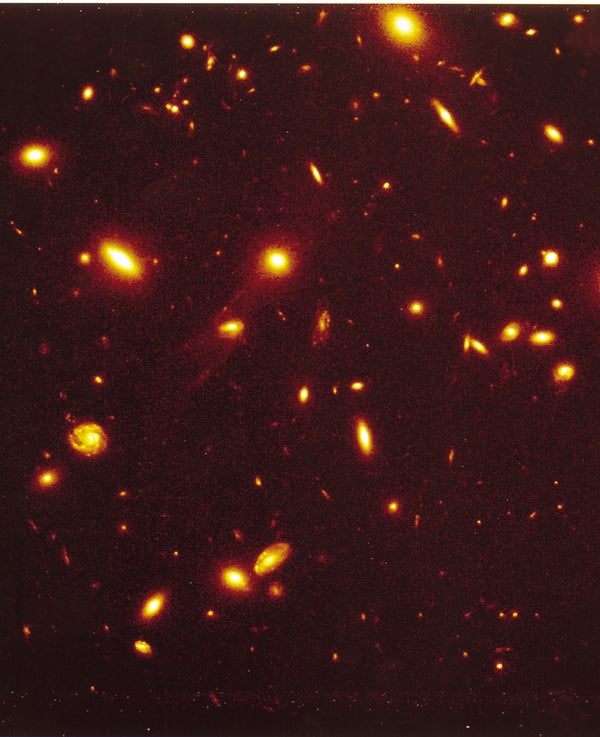
GALAXY CLUSTER is representative of what the universe looked like when it was 60 percent of its present age. The Hubble Space Telescope captured the image by focusing on the cluster as it completed 10 orbits. This image is one of the longest and clearest exposures ever produced. Several pairs of galaxies appear to be caught in one another’s gravitational field. Such interactions are rarely found in nearby clusters and are evidence that the universe is evolving.
Editor’s Note (10/8/19): Cosmologist James Peebles won a 2019 Nobel Prize in Physics for his contributions to theories of how our universe began and evolved. He describes these ideas in this article, which he co-wrote for Scientific American in 1994.
At a particular instant roughly 15 billion years ago, all the matter and energy we can observe, concentrated in a region smaller than a dime, began to expand and cool at an incredibly rapid rate. By the time the temperature had dropped to 100 million times that of the sun’s core, the forces of nature assumed their present properties, and the elementary particles known as quarks roamed freely in a sea of energy. When the universe had expanded an additional 1,000 times, all the matter we can measure filled a region the size of the solar system.
At that time, the free quarks became confined in neutrons and protons. After the universe had grown by another factor of 1,000, protons and neutrons combined to form atomic nuclei, including most of the helium and deuterium present today. All of this occurred within the first minute of the expansion. Conditions were still too hot, however, for atomic nuclei to capture electrons. Neutral atoms appeared in abundance only after the expansion had continued for 300,000 years and the universe was 1,000 times smaller than it is now. The neutral atoms then began to coalesce into gas clouds, which later evolved into stars. By the time the universe had expanded to one fifth its present size, the stars had formed groups recognizable as young galaxies.
On supporting science journalism
If you're enjoying this article, consider supporting our award-winning journalism by subscribing . By purchasing a subscription you are helping to ensure the future of impactful stories about the discoveries and ideas shaping our world today.
When the universe was half its present size, nuclear reactions in stars had produced most of the heavy elements from which terrestrial planets were made. Our solar system is relatively young: it formed five billion years ago, when the universe was two thirds its present size. Over time the formation of stars has consumed the supply of gas in galaxies, and hence the population of stars is waning. Fifteen billion years from now stars like our sun will be relatively rare, making the universe a far less hospitable place for observers like us.
Our understanding of the genesis and evolution of the universe is one of the great achievements of 20th-century science. This knowledge comes from decades of innovative experiments and theories. Modern telescopes on the ground and in space detect the light from galaxies billions of light-years away, showing us what the universe looked like when it was young. Particle accelerators probe the basic physics of the high-energy environment of the early universe. Satellites detect the cosmic background radiation left over from the early stages of expansion, providing an image of the universe on the largest scales we can observe.
Our best efforts to explain this wealth of data are embodied in a theory known as the standard cosmological model or the big bang cosmology. The major claim of the theory is that in the largescale average the universe is expanding in a nearly homogeneous way from a dense early state. At present, there are no fundamental challenges to the big bang theory, although there are certainly unresolved issues within the theory itself. Astronomers are not sure, for example, how the galaxies were formed, but there is no reason to think the process did not occur within the framework of the big bang. Indeed, the predictions of the theory have survived all tests to date.
Yet the big bang model goes only so far, and many fundamental mysteries remain. What was the universe like before it was expanding? (No observation we have made allows us to look back beyond the moment at which the expansion began.) What will happen in the distant future, when the last of the stars exhaust the supply of nuclear fuel? No one knows the answers yet.
Our universe may be viewed in many lights—by mystics, theologians, philosophers or scientists. In science we adopt the plodding route: we accept only what is tested by experiment or observation. Albert Einstein gave us the now well-tested and accepted Theory of General Relativity, which establishes the relations between mass, energy, space and time. Einstein showed that a homogeneous distribution of matter in space fits nicely with his theory. He assumed without discussion that the universe is static, unchanging in the large-scale average [see “How Cosmology Became a Science,” by Stephen G. Brush; SCIENTIFIC AMERICAN, August 1992].
In 1922 the Russian theorist Alexander A. Friedmann realized that Einstein’s universe is unstable; the slightest perturbation would cause it to expand or contract. At that time, Vesto M. Slipher of Lowell Observatory was collecting the first evidence that galaxies are actually moving apart. Then, in 1929, the eminent astronomer Edwin P. Hubble showed that the rate a galaxy is moving away from us is roughly proportional to its distance from us.
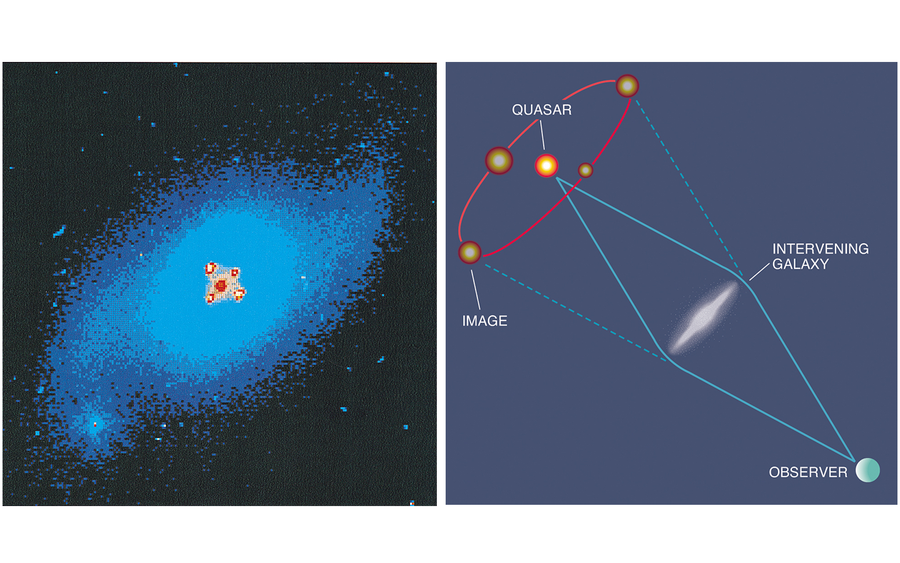
MULTIPLE IMAGES of a distant quasar ( left ) are the result of an effect known as gravitational lensing. The effect occurs when light from a distant object is bent by the gravitational field of an intervening galaxy. In this case, the galaxy, which is visible in the center, produces four images of the quasar. The photograph was produced using the Hubble telescope.
The existence of an expanding universe implies that the cosmos has evolved from a dense concentration of matter into the present broadly spread distribution of galaxies. Fred Hoyle, an English cosmologist, was the first to call this process the big bang. Hoyle intended to disparage the theory, but the name was so catchy it gained popularity. It is somewhat misleading, however, to describe the expansion as some type of explosion of matter away from some particular point in space.
That is not the picture at all: in Einstein’s universe the concept of space and the distribution of matter are intimately linked; the observed expansion of the system of galaxies reveals the unfolding of space itself. An essential feature of the theory is that the average density in space declines as the universe expands; the distribution of matter forms no observable edge. In an explosion the fastest particles move out into empty space, but in the big bang cosmology, particles uniformly fill all space. The expansion of the universe has had little influence on the size of galaxies or even clusters of galaxies that are bound by gravity; space is simply opening up between them. In this sense, the expansion is similar to a rising loaf of raisin bread. The dough is analogous to space, and the raisins, to clusters of galaxies. As the dough expands, the raisins move apart. Moreover, the speed with which any two raisins move apart is directly and positively related to the amount of dough separating them.
The evidence for the expansion of the universe has been accumulating for some 60 years. The first important clue is the redshift. A galaxy emits or absorbs some wavelengths of light more strongly than others. If the galaxy is moving away from us, these emission and absorption features are shifted to longer wavelengths—that is, they become redder as the recession velocity increases. This phenomenon is known as the redshift.
Hubble’s measurements indicated that the redshift of a distant galaxy is greater than that of one closer to the earth. This relation, now known as Hubble’s law, is just what one would expect in a uniformly expanding universe. Hubble’s law says the recession velocity of a galaxy is equal to its distance multiplied by a quantity called Hubble’s constant. The redshift effect in nearby galaxies is relatively subtle, requiring good instrumentation to detect it. In contrast, the redshift of very distant objects—radio galaxies and quasars—is an awesome phenomenon; some appear to be moving away at greater than 90 percent of the speed of light.
Hubble contributed to another crucial part of the picture. He counted the number of visible galaxies in different directions in the sky and found that they appear to be rather uniformly distributed. The value of Hubble’s constant seemed to be the same in all directions, a necessary consequence of uniform expansion. Modern surveys confirm the fundamental tenet that the universe is homogeneous on large scales. Although maps of the distribution of the nearby galaxies display clumpiness, deeper surveys reveal considerable uniformity.
The Milky Way, for instance, resides in a knot of two dozen galaxies; these in turn are part of a complex of galaxies that protrudes from the so-called local supercluster. The hierarchy of clustering has been traced up to dimensions of about 500 million light-years. The fluctuations in the average density of matter diminish as the scale of the structure being investigated increases. In maps that cover distances that reach close to the observable limit, the average density of matter changes by less than a tenth of a percent.
To test Hubble’s law, astronomers need to measure distances to galaxies. One method for gauging distance is to observe the apparent brightness of a galaxy. If one galaxy is four times fainter in the night sky than an otherwise comparable galaxy, then it can be estimated to be twice as far away. This expectation has now been tested over the whole of the visible range of distances.
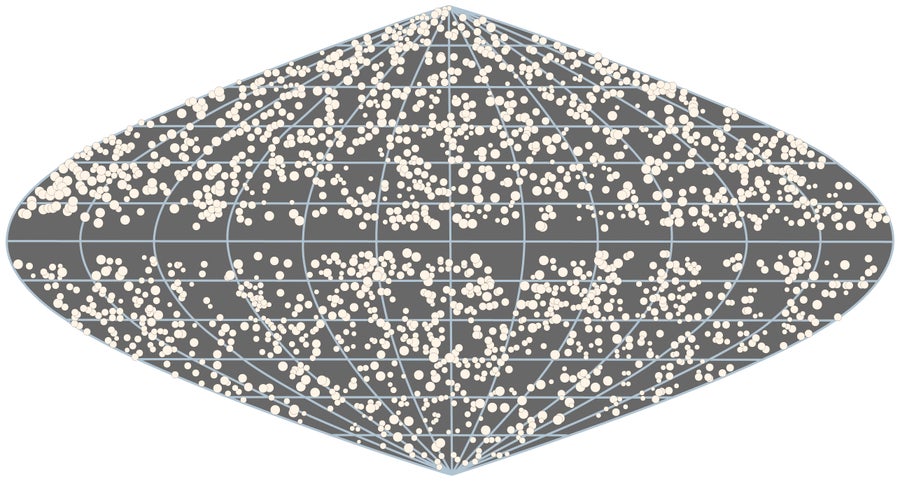
HOMOGENEOUS DISTRIBUTION of galaxies is apparent in a map that includes objects from 300 to 1,000 million light-years away. The only inhomogeneity, a gap near the center line, occurs because part of the sky is obscured by the Milky Way. Michael Strauss of the Institute for Advanced Study in Princeton, N.J., created the map using data from NASA’s Infrared Astronomical Satellite .
Some critics of the theory have pointed out that a galaxy that appears to be smaller and fainter might not actually be more distant. Fortunately, there is a direct indication that objects whose redshifts are larger really are more distant. The evidence comes from observations of an effect known as gravitational lensing. An object as massive and compact as a galaxy can act as a crude lens, producing a distorted, magnified image (or even many images) of any background radiation source that lies behind it. Such an object does so by bending the paths of light rays and other electromagnetic radiation. So if a galaxy sits in the line of sight between the earth and some distant object, it will bend the light rays from the object so that they are observable [see “Gravitational Lenses,” by Edwin L. Turner; SCIENTIFIC AMERICAN, July 1988]. During the past decade, astronomers have discovered more than a dozen gravitational lenses. The object behind the lens is always found to have a higher redshift than the lens itself, confirming the qualitative prediction of Hubble’s law.
Hubble’s law has great significance not only because it describes the expansion of the universe but also because it can be used to calculate the age of the cosmos. To be precise, the time elapsed since the big bang is a function of the present value of Hubble’s constant and its rate of change. Astronomers have determined the approximate rate of the expansion, but no one has yet been able to measure the second value precisely.
Still, one can estimate this quantity from knowledge of the universe’s average density. One expects that because gravity exerts a force that opposes expansion, galaxies would tend to move apart more slowly now than they did in the past. The rate of change in expansion is therefore related to the gravitational pull of the universe set by its average density. If the density is that of just the visible material in and around galaxies, the age of the universe probably lies between 12 and 20 billion years. (The range allows for the uncertainty in the rate of expansion.)
Yet many researchers believe the density is greater than this minimum value. So-called dark matter would make up the difference. A strongly defended argument holds that the universe is just dense enough that in the remote future the expansion will slow almost to zero. Under this assumption, the age of the universe decreases to the range of seven to 13 billion years.
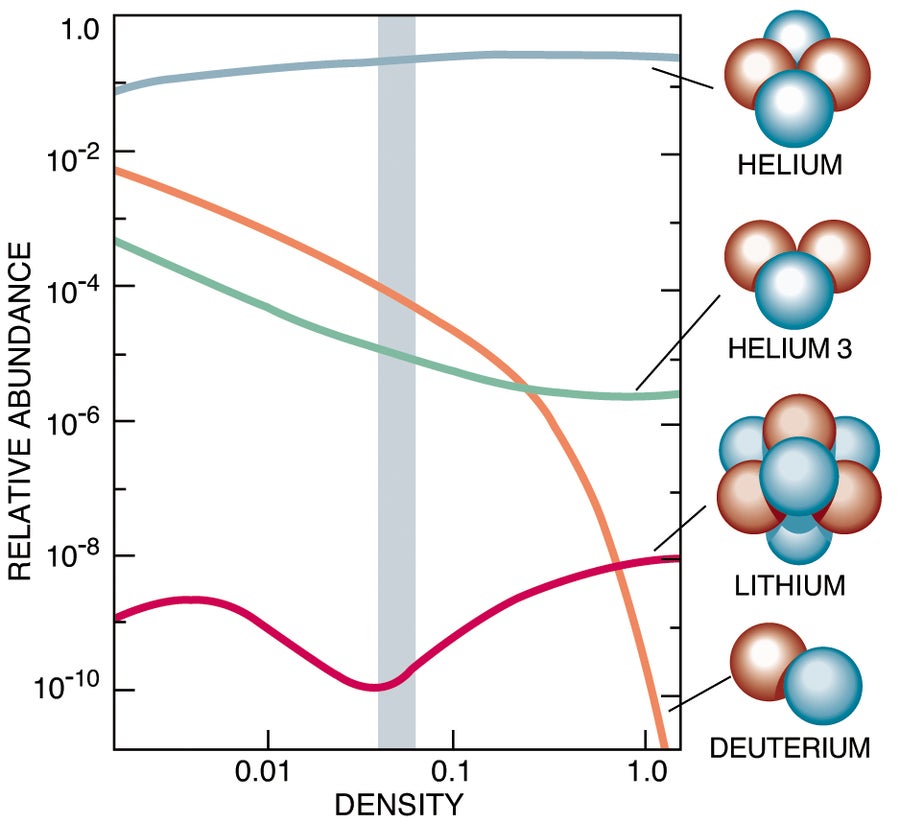
DENSITY of neutrons and protons in the universe determined the abundances of certain elements. For a higher density universe, the computed helium abundance is little different, and the computed abundance of deuterium is considerably lower. The shaded region is consistent with the observations, ranging from an abundance of 24 percent for helium to one part in 1010 for the lithium isotope. This quantitative agreement is a prime success of the big bang cosmology.
To improve these estimates, many astronomers are involved in intensive research to measure both the distances to galaxies and the density of the universe. Estimates of the expansion time provide an important test for the big bang model of the universe. If the theory is correct, everything in the visible universe should be younger than the expansion time computed from Hubble’s law.
These two timescales do appear to be in at least rough concordance. For example, the oldest stars in the disk of the Milky Way galaxy are about nine billion years old—an estimate derived from the rate of cooling of white dwarf stars. The stars in the halo of the Milky Way are somewhat older, about 15 billion years—a value derived from the rate of nuclear fuel consumption in the cores of these stars. The ages of the oldest known chemical elements are also approximately 15 billion years—a number that comes from radioactive dating techniques. Workers in laboratories have derived these age estimates from atomic and nuclear physics. It is noteworthy that their results agree, at least approximately, with the age that astronomers have derived by measuring cosmic expansion.
Another theory, the steady state theory, also succeeds in accounting for the expansion and homogeneity of the universe. In 1946 three physicists in England—Hoyle, Hermann Bondi and Thomas Gold—proposed such a cosmology. In their theory the universe is forever expanding, and matter is created spontaneously to fill the voids. As this material accumulates, they suggested, it forms new stars to replace the old. This steady state hypothesis predicts that ensembles of galaxies close to us should look statistically the same as those far away. The big bang cosmology makes a different prediction: if galaxies were all formed long ago, distant galaxies should look younger than those nearby because light from them requires a longer time to reach us. Such galaxies should contain more shortlived stars and more gas out of which future generations of stars will form.
The test is simple conceptually, but it took decades for astronomers to develop detectors sensitive enough to study distant galaxies in detail. When astronomers examine nearby galaxies that are powerful emitters of radio wavelengths, they see, at optical wavelengths, relatively round systems of stars. Distant radio galaxies, on the other hand, appear to have elongated and sometimes irregular structures. Moreover, in most distant radio galaxies, unlike the ones nearby, the distribution of light tends to be aligned with the pattern of the radio emission.
Likewise, when astronomers study the population of massive, dense clusters of galaxies, they find differences between those that are close and those far away. Distant clusters contain bluish galaxies that show evidence of ongoing star formation. Similar clusters that are nearby contain reddish galaxies in which active star formation ceased long ago. Observations made with the Hubble Space Telescope confirm that at least some of the enhanced star formation in these younger clusters may be the result of collisions between their member galaxies, a process that is much rarer in the present epoch.

DISTANT GALAXIES differ greatly from those nearby—an observation that shows that galaxies evolved from earlier, more irregular forms. Among galaxies that are bright at both optical ( blue ) and radio ( red ) wavelengths, the nearby galaxies tend to have smooth elliptical shapes at optical wavelengths and very elongated radio images. As redshift, and therefore distance, increases, galaxies have more irregular elongated forms that appear aligned at optical and radio wavelengths. The galaxy at the far right is seen as it was at 10 percent of the present age of the universe. The images were assembled by Pat McCarthy of the Carnegie Institute.
So if galaxies are all moving away from one another and are evolving from earlier forms, it seems logical that they were once crowded together in some dense sea of matter and energy. Indeed, in 1927, before much was known about distant galaxies, a Belgian cosmologist and priest, Georges Lemaître, proposed that the expansion of the universe might be traced to an exceedingly dense state he called the primeval “super-atom.” It might even be possible, he thought, to detect remnant radiation from the primeval atom. But what would this radiation signature look like?
When the universe was very young and hot, radiation could not travel very far without being absorbed and emitted by some particle. This continuous exchange of energy maintained a state of thermal equilibrium; any particular region was unlikely to be much hotter or cooler than the average. When matter and energy settle to such a state, the result is a so-called thermal spectrum, where the intensity of radiation at each wavelength is a definite function of the temperature. Hence, radiation originating in the hot big bang is recognizable by its spectrum.
In fact, this thermal cosmic background radiation has been detected. While working on the development of radar in the 1940s, Robert H. Dicke, then at the Massachusetts Institute of Technology, invented the microwave radiometer—a device capable of detecting low levels of radiation. In the 1960s Bell Laboratories used a radiometer in a telescope that would track the early communications satellites Echo-1 and Telstar. The engineer who built this instrument found that it was detecting unexpected radiation. Arno A. Penzias and Robert W. Wilson identified the signal as the cosmic background radiation. It is interesting that Penzias and Wilson were led to this idea by the news that Dicke had suggested that one ought to use a radiometer to search for the cosmic background.
Astronomers have studied this radiation in great detail using the Cosmic Background Explorer (COBE) satellite and a number of rocket-launched, balloon-borne and ground-based experiments. The cosmic background radiation has two distinctive properties. First, it is nearly the same in all directions. (As George F. Smoot of Lawrence Berkeley Laboratory and his team discovered in 1992, the variation is just one part per 100,000.) The interpretation is that the radiation uniformly fills space, as predicted in the big bang cosmology. Second, the spectrum is very close to that of an object in thermal equilibrium at 2.726 kelvins above absolute zero. To be sure, the cosmic background radiation was produced when the universe was far hotter than 2.726 degrees, yet researchers anticipated correctly that the apparent temperature of the radiation would be low. In the 1930s Richard C. Tolman of the California Institute of Technology showed that the temperature of the cosmic background would diminish because of the universe’s expansion.
The cosmic background radiation provides direct evidence that the universe did expand from a dense, hot state, for this is the condition needed to produce the radiation. In the dense, hot early universe thermonuclear reactions produced elements heavier than hydrogen, including deuterium, helium and lithium. It is striking that the computed mix of the light elements agrees with the observed abundances. That is, all evidence indicates that the light elements were produced in the hot, young universe, whereas the heavier elements appeared later, as products of the thermonuclear reactions that power stars.
The theory for the origin of the light elements emerged from the burst of research that followed the end of World War II. George Gamow and graduate student Ralph A. Alpher of George Washington University and Robert Herman of the Johns Hopkins University Applied Physics Laboratory and others used nuclear physics data from the war e›ort to predict what kind of nuclear processes might have occurred in the early universe and what elements might have been produced. Alpher and Herman also realized that a remnant of the original expansion would still be detectable in the existing universe.
Despite the fact that significant details of this pioneering work were in error, it forged a link between nuclear physics and cosmology. The workers demonstrated that the early universe could be viewed as a type of thermonuclear reactor. As a result, physicists have now precisely calculated the abundances of light elements produced in the big bang and how those quantities have changed because of subsequent events in the interstellar medium and nuclear processes in stars.
Our grasp of the conditions that prevailed in the early universe does not translate into a full understanding of how galaxies formed. Nevertheless, we do have quite a few pieces of the puzzle. Gravity causes the growth of density fluctuations in the distribution of matter, because it more strongly slows the expansion of denser regions, making them grow still denser. This process is observed in the growth of nearby clusters of galaxies, and the galaxies themselves were probably assembled by the same process on a smaller scale.
The growth of structure in the early universe was prevented by radiation pressure, but that changed when the universe had expanded to about 0.1 percent of its present size. At that point, the temperature was about 3,000 kelvins, cool enough to allow the ions and electrons to combine to form neutral hydrogen and helium. The neutral matter was able to slip through the radiation and to form gas clouds that could collapse to star clusters. Observations show that by the time the universe was one fifth its present size, matter had gathered into gas clouds large enough to be called young galaxies.
A pressing challenge now is to reconcile the apparent uniformity of the early universe with the lumpy distribution of galaxies in the present universe. Astronomers know that the density of the early universe did not vary by much, because they observe only slight irregularities in the cosmic background radiation. So far it has been easy to develop theories that are consistent with the available measurements, but more critical tests are in progress. In particular, different theories for galaxy formation predict quite different fluctuations in the cosmic background radiation on angular scales less than about one degree. Measurements of such tiny fluctuations have not yet been done, but they might be accomplished in the generation of experiments now under way. It will be exciting to learn whether any of the theories of galaxy formation now under consideration survive these tests.
The present-day universe has provided ample opportunity for the development of life as we know it—there are some 100 billion billion stars similar to the sun in the part of the universe we can observe. The big bang cosmology implies, however, that life is possible only for a bounded span of time: the universe was too hot in the distant past, and it has limited resources for the future. Most galaxies are still producing new stars, but many others have already exhausted their supply of gas. Thirty billion years from now, galaxies will be much darker and filled with dead or dying stars, so there will be far fewer planets capable of supporting life as it now exists.
The universe may expand forever, in which case all the galaxies and stars will eventually grow dark and cold. The alternative to this big chill is a big crunch. If the mass of the universe is large enough, gravity will eventually reverse the expansion, and all matter and energy will be reunited. During the next decade, as researchers improve techniques for measuring the mass of the universe, we may learn whether the present expansion is headed toward a big chill or a big crunch.
In the near future, we expect new experiments to provide a better understanding of the big bang. As we improve measurements of the expansion rate and the ages of stars, we may be able to confirm that the stars are indeed younger than the expanding universe. The larger telescopes recently completed or under construction may allow us to see how the mass of the universe affects the curvature of spacetime, which in turn influences our observations of distant galaxies.
We will also continue to study issues that the big bang cosmology does not address. We do not know why there was a big bang or what may have existed before. We do not know whether our universe has siblings—other expanding regions well removed from what we can observe. We do not understand why the fundamental constants of nature have the values they do. Advances in particle physics suggest some interesting ways these questions might be answered; the challenge is to find experimental tests of the ideas.
In following the debate on such matters of cosmology, one should bear in mind that all physical theories are approximations of reality that can fail if pushed too far. Physical science advances by incorporating earlier theories that are experimentally supported into larger, more encompassing frameworks. The big bang theory is supported by a wealth of evidence: it explains the cosmic background radiation, the abundances of light elements and the Hubble expansion. Thus, any new cosmology surely will include the big bang picture. Whatever developments the coming decades may bring, cosmology has moved from a branch of philosophy to a physical science where hypotheses meet the test of observation and experiment.
Plane Symmetric Action of the Universe in \(f\left( {R, L_{m} } \right)\) Gravity
- Original Paper
- Published: 20 November 2024
- Volume 10 , article number 180 , ( 2024 )
Cite this article
- S. N. Bayaskar 1 &
- Anuja A. Dhanagare 1
In this paper, we have studied plane symmetric cosmological model with the isotropic matter distribution. This is done under the framework of \(f\left( {R,L_{m} } \right)\) gravity theory. This modified gravity theory, represented by \(f\left( {R,L_{m} } \right) = \frac{R}{2} + L_{m}^{\alpha } + \beta\) , which is a combination of both the Ricci scalar (R) and matter Lagrangian density \((L_{m} )\) , we consider \(L_{m} = \rho\) . Our goal was to analyse the behaviour of the expansion rate of the universe over time. We solved the field equation by assuming (i) the constant deceleration parameter (DP) proposed by Berman, i.e. q = constant. (ii) a time varying deceleration parameter \(q\left( t \right) = - 1 + \frac{n\zeta }{{\left( {\zeta + t} \right)^{2} }}\) , which yields \(a\left( t \right) = \left[ {t^{\zeta } e^{t} } \right]^{\frac{1}{n}}\) .We discussed the physical properties of the models. Additionally, we use the equation of state (EoS) parameter, the jerk parameter and the statefinder parameters as analytical tools to understand the evolution of the Universe in this modified gravity framework. This research offers significant insights into the anisotropic behaviour of the Universe within the framework of \(f\left( {R,L_{m} } \right)\) gravity, enhancing our comprehension of the fundamental dynamics of cosmic evolution.
This is a preview of subscription content, log in via an institution to check access.
Access this article
Subscribe and save.
- Get 10 units per month
- Download Article/Chapter or eBook
- 1 Unit = 1 Article or 1 Chapter
- Cancel anytime
Price includes VAT (Russian Federation)
Instant access to the full article PDF.
Rent this article via DeepDyve
Institutional subscriptions
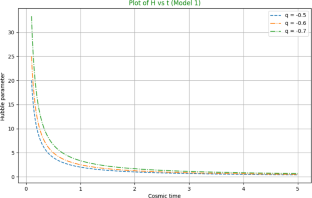
Data Availability
No datasets were generated or analysed during the current study.
Bennett, C.L., Banday, A.J., Gorski, K.M.: ApJ 464 , L1 (1996)
Article Google Scholar
de Bernardis, P., de Bocketal, P.A.R.A.J.J.: BOOMERanG Collaboration. AIP Conf. Proc. 555 , 85 (2001)
Google Scholar
Mason, B.S., et al.: ApJ 591 , 540 (2003)
Riess, A.G., et al.: Astrophys. J. 116 , 1009 (1998)
Perlmutter, S., et al.: Nature 391 , 51 (1998)
Perlmutter, S., et al.: Astrophys. J. 517 , 565 (1999)
Riess, A.G., et al.: Astrophys. J. 607 , 665–687 (2004)
Eisenstein, D.J., et al.: Astrophys. J. 633 , 560 (2005)
Percival, W.J., et al.: Mon. Not. R. Astron. Soc. 401 , 2148 (2010)
Spergel, D.N., et al.: Astrophys. J. Suppl. Ser. 148 , 175–194 (2003)
Aghanim, N., Akrami, Y., Ashdown, M., et al.: A&A 641 , A6 (2020)
Koivisto, T., Mota, D.F.: Phys. Rev. D 73 , 083502 (2006)
Article MathSciNet Google Scholar
Daniel, S.F.: Phys. Rev. D 77 , 103513 (2008)
Bennett, C.L., et al.: Astrophys. J. Suppl. Ser. 148 , 1–27 (2003)
Caldwell, R.R., Doran, M.: Phys. Rev. D 69 , 103517 (2004)
Buchdahl, H.A.: Mon. Not. R. Astron. Soc. 150 , 1 (1970)
Kerner, R.: Gen. Relativity. Gravit. 14 , 453 (1982)
Kleinert, H., Schmidt, H.J.: Gen. Relat. Gravit. 34 , 1295 (2002)
Nojiri, S., Odintsov, S.D.: Phys. Lett. B 657 , 238 (2007)
Nojiri, S., Odintsov, S.D.: Phys. Rev. D 77 , 026007 (2008)
Cognola, G., et al.: Phys. Rev. D 77 , 046009 (2008)
Bertolami, O., et al.: Phys. Rev. D 75 , 104016 (2007)
Harko, T.: Phys. Lett. B 669 , 376 (2008)
Harko, T.: Phys. Rev. D 81 , 084050 (2010)
Harko, T.: Phys. Rev. D 81 , 044021 (2010)
Faraoni, V.: Phys. Rev. D 76 , 127501 (2007)
Faraoni, V.: Phys. Rev. D 80 , 124040 (2009)
Harko, T., Lobo, F.S.N.: Eur. Phys. J. C 70 , 373–379 (2010)
Wang, J., Liao, K.: Class. Quantum Gravity 29 , 215016 (2012)
Goncalves, B.S., Moraes, P.H.R.S.: Fortsch. Phys. 71 , 2200153 (2023)
Jaybhaye, L.V., Solanki, R., Mandal, S., Sahoo, P.K.: Phys. Lett. B 831 , 137148 (2022)
Jaybhaye, L.V., Bhattacharjee, S., Sahoo, P.K.: Phys. Dark Uni. 40 , 101223 (2023)
Jaybhaye, L.V., Mandal, S., Sahoo, P.K.: IJGMMP 19 , 04 (2022)
Pawde, J.L., Mapari, R., Patil, V., Pawar, D.: Eur. Phys. J. C 84 , 320 (2024)
Kavya, N.S., Venkatesha, V., Mandal, S., Sahoo, P.K.: Phys. Dark Uni 38 , 101126 (2022)
Solanki, R., Hassan, Z., Sahoo, P.K.: Chin. J. Phys. 85 , 74–88 (2023)
Kavya, N.S., Venkatesha, V., Mustafa, G., Sahoo, P.K.: Chin. J. of Phys. 84 , 1–11 (2023)
Patil, V., Pawde, J., Mapari, R., Waghmare, S.: East Eur. J. Phys. 4 , 8 (2023). https://doi.org/10.26565/2312-4334-2023-4-01
Bayaskar, S.N., Pawar, D.D., Deshmukh, A.G.: Rom. J. Phys. 54 (7–8), 763–770 (2009)
Pawar, D.D., Bayaskar, S.N., Patil, V.R.: Bulg. J. Phys. 36 , 68–75 (2009)
Thakare, V.A., Mapari, R.V., Thakre, S.S.: East Euro J. Phys. 3 , 108–121 (2023). https://doi.org/10.26565/2312-4334-2023-3-08
Shaikh, A.Y., Bhoyar, S.R.: Prespacetime J. 6 (11), 1179–1197 (2015)
Shaikh, A.Y., Shaikh, A.S.A.Y., Wankhade, K.S.: Pramana J. Phys. Phys. 95 , 19 (2021). https://doi.org/10.1007/s12043-020-02047-z
Mapari, R.V.; Pawar, D.D.; Patil, V.R.; Pawde, J.L.: arXiv preprint https://arxiv.org/abs/2403.00882 . (2024).
Berman, M.S.: Nuovo Cimento B 74 , 182–186 (1983)
Berman, M.S., Gomide, F.M.: Gen. Relat. Gravit. 20191 , 198 (1988)
Johri, V.B., Desikan, K.: Pramana 42 , 473–481 (1994)
Johri, V.B., Desikan, K.: Gen. Relativ. Gravit. 26 , 1217–1232 (1994)
Singh, G.P., Desikan, K.: Pramana 49 , 205–212 (1997)
Pradhan, A., Yadav, V.K., Chakrabarty, I.: Inter. J. Mod. Phys. D 10 , 339–350 (2001)
Pradhan, A., Vishwakarma, A.K.: Inter. J. Mod. Phys. D 11 , 1195–1208 (2002)
Pradhan, A., Aotemshi, I.: Int. J. Mod. Phys. D 11 , 1419–1434 (2002)
Saha, B., Rikhvitsky, V.: Physica D 219 , 168–176 (2006)
Saha, B.: Astrophys. Space Sci. 302 , 83–91 (2006)
Singh, C.P., Kumar, S.: Int. J. Mod. Phys. D 15 , 419 (2006)
Singh, C.P., Kumar, S.: Astrophys. Space Sci. 310 , 31–39 (2007)
Singh, C.P.: Pramana Phys. Astron. 68 , 707–720 (2007)
Reddy, D.R.K., Naidu, R.L., Rao, V.U.M.: Int. J. Theor. Phys. 46 , 1443–1448 (2007)
Reddy, D.R.K., Naidu, R.L., Adhav, K.S.: Astrophys. Space Sci. 307 , 365–367 (2007)
Singh, J.P., Baghel, P.S.: Int. J. Theo. Phys. 48 , 449–462 (2009)
Akarsu, O., Kilinc, C.B.: Gen. Relativ. Gravit. 42 , 119–140 (2010)
Pradhan, A., Singh, A.K.: Int. J. Theor. Phys. 50 , 916–933 (2011)
Pradhan, A., Amirhaschi, H., Saha, B.: Int. J. Theor. Phys. 50 , 2923–2938 (2011)
Ghate, H.R., Sontakke, A.S.: Prespacetime J. 4 , 366–376 (2013)
Ghate, H.R., Sontakke, A.S.: Math. Sci. Int. Res. J. 3 , 46–53 (2014)
Pradhan, A., Shahi, J.P., Singh, C.B.: Braz. J. Phys. 36 , 1227–1231 (2006)
Tripathi, S.K., Nigam, S.K., Kumar, S., Sharma, P.K.: Int. J. Phys. Math. Sci. 2 , 53–57 (2012)
Chawla, C., Mishra, R.K., Pradhan, A.: Rom. J. Phys. 58 , 1000–1013 (2013)
Yadav, A.K. Anisotropic Models of Accelerating Universe, 80–98 (2011).
Akarsu, Ö., Dereli, T.: Int. J. Theor. Phys. 51 , 612–621 (2012)
Mishra, R.K., Dua, H., Chand, A.: Astrophys. Space Sci. 363 , 1–8 (2018)
Mishra, R.K., Chand, A.: Astrophys. Space Sci. 362 (8), 11 (2017)
Mishra, R.K., Chand, A., Pradhan, A.: Int. J. Theor. Phys. 55 , 1241–1256 (2016)
Mishra, R.K., Chand, A.: Astrophys. Space Sci. 361 , 259 (2016)
Chawla, C., Mishra, R.K.: Rom. Reports Phys. 58 , 75–85 (2013)
Adhav, K.S.: Int. J. Math. Arch. 2 , 2149–2156 (2011)
Adhav, K.S.: Eur. Phys. J. Plus 126 , 122–127 (2011)
Akarsu, O., Dereli, T., Kumar, S., Xu, L.: The Eur. Phy. J. Plus 129 , 22–36 (2014)
Saha, B., Amirhashchi, H., Pradhan, A.: Astrophys. Space Sci. 342 , 257–267 (2012)
Pradhan, A., Amirhashchi, H.: Mod. Phys. Lett. A 26 , 2261–2275 (2011)
Lohakare, S.V., Tripathy, S.K., Mishra, B.: Cosmological model with time varying deceleration parameter in F (R, G) gravity. Phys. Scr. 96 (12), 125039 (2021)
Yadav, A.K.: Res. Astron. Astrophys. 12 , 1467–1474 (2012)
Yadav, A.K.: Chin. Phys. Lett. 29 , 079801 (2012)
Pradhan, A., Singh, A.K., Chouhan, D.S.: Int. J. Theor. Phys. 52 , 266–278 (2013)
Ghate, H.R., Sontakke, A.S., Patil, Y.D.: Int. J. Astron. Astrophys. 5 , 302–323 (2015). https://doi.org/10.4236/ijaa.2015.54033
Harko, T., Lobo, F.S.N.: Galaxies 2014 (2), 410–465 (2014)
Harko, T., et al.: Eur. Phys. J. C 75 , 386 (2015)
Download references
This research was conducted without any financial support from any funding agencies or organizations.
Author information
Authors and affiliations.
Department of Mathematics, Adarsha Science, J. B. Art and Birla Commerce College, Dhamangaon Rly, Dist., Amravati, 444 709, India
S. N. Bayaskar & Anuja A. Dhanagare
You can also search for this author in PubMed Google Scholar
Contributions
Anuja A. Dhanagare wrote the main manuscript of the paper, solved all the equations and plot the graphs. S. N. Bayaskar discussed all the properties of the model. We both reviewed the manuscript.
Corresponding author
Correspondence to Anuja A. Dhanagare .
Ethics declarations
Competing interests.
The authors declare no competing interests.
Additional information
Publisher's note.
Springer Nature remains neutral with regard to jurisdictional claims in published maps and institutional affiliations.
Rights and permissions
Springer Nature or its licensor (e.g. a society or other partner) holds exclusive rights to this article under a publishing agreement with the author(s) or other rightsholder(s); author self-archiving of the accepted manuscript version of this article is solely governed by the terms of such publishing agreement and applicable law.
Reprints and permissions
About this article
Bayaskar, S.N., Dhanagare, A.A. Plane Symmetric Action of the Universe in \(f\left( {R, L_{m} } \right)\) Gravity. Int. J. Appl. Comput. Math 10 , 180 (2024). https://doi.org/10.1007/s40819-024-01812-7
Download citation
Accepted : 01 November 2024
Published : 20 November 2024
DOI : https://doi.org/10.1007/s40819-024-01812-7
Share this article
Anyone you share the following link with will be able to read this content:
Sorry, a shareable link is not currently available for this article.
Provided by the Springer Nature SharedIt content-sharing initiative
- \(f\left( {R,L_{m} } \right)\) gravity
- Plane symmetric metric
- Perfect fluid
- Constant deceleration parameter
- Time varying deceleration parameter
- Find a journal
- Publish with us
- Track your research
Thank you for visiting nature.com. You are using a browser version with limited support for CSS. To obtain the best experience, we recommend you use a more up to date browser (or turn off compatibility mode in Internet Explorer). In the meantime, to ensure continued support, we are displaying the site without styles and JavaScript.
- View all journals
Cosmology articles from across Nature Portfolio
Cosmology is the study of the universe; its birth, evolution, and ultimate fate. This includes further developing and refining the prevailing model, the Big Bang theory, investigating the universe’s rate of expansion, and measuring radiation left over from the Big Bang, the so-called cosmic microwave background.
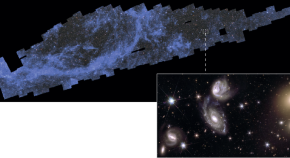
The first piece of Euclid’s cosmic puzzle
- Lindsay Oldham
Latest Research and Reviews
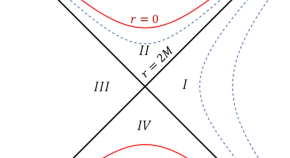
Explicit construction of Penrose diagrams for black hole to white hole transition with spacelike thin shells
- Wei-Chen Lin
- Dejan Stojkovic
- Dong-han Yeom
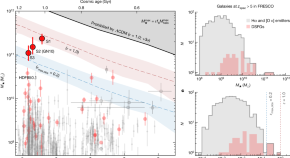

Accelerated formation of ultra-massive galaxies in the first billion years
A study of 36 massive galaxies at redshifts between 5 and 9 from the JWST FRESCO survey finds that galaxy formation of the most massive galaxies is 2–3 times higher than the most efficient galaxies at later epochs.
- Mengyuan Xiao
- Pascal A. Oesch
- J. Stuart B. Wyithe
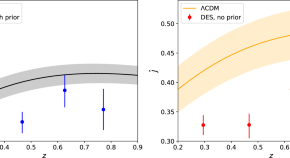
Measurement of the Weyl potential evolution from the first three years of dark energy survey data
The Weyl potential provides a direct way of testing the theory of gravity and the validity of the Λ CDM model. Here, the authors show Weyl potential measured at four redshifts bins and find that the measured values in the two lowest bins are in mild tension with the Λ CDM predictions.
- Isaac Tutusaus
- Camille Bonvin
- Nastassia Grimm
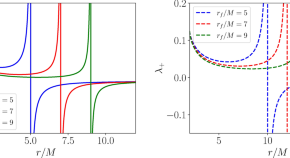
Event Horizon Telescope observations exclude compact objects in baseline mimetic gravity
- Mohsen Khodadi
- Sunny Vagnozzi
- Javad T. Firouzjaee
Generalized redshift formula through an energy-based framework
- F. Ibarra-Castor
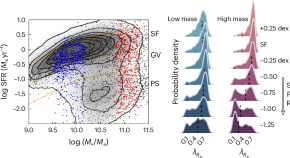
Universal bimodality in kinematic morphology and the divergent pathways to galaxy quenching
Beneath the superficial diversity and complexity of galaxy morphology in our local Universe, galaxies show underlying simplicity and have universally bimodal distributions of normalized specific angular momentum of stars, revealed by MaNGA observations.
- Yingjie Peng
- Michele Cappellari
News and Comment

The span of space
Twinkle, twinkle little star, tell me just how far you are. Richard I. Anderson discusses standard candles and their applications.
- Richard I. Anderson

Gravitational wells in the large-scale structure of the Universe
The Universe comprises clusters and voids of galaxies and has been shaped by gravity over 13 billion years. Hamiltonian Monte Carlo simulations constrained by observed positions and velocities of galaxies produce maps of the density and velocity field of matter in the local Universe, revealing immense gravitational wells, which can inform future theories of structure formation.
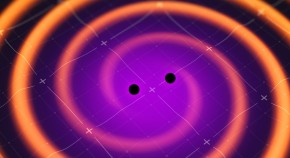
Five new ways to catch gravitational waves — and the secrets they’ll reveal
Observatories, experiments and techniques are being developed to spot ripples in space-time at frequencies that currently can’t be detected.
- Davide Castelvecchi
Distance measurement by machine learning
- Morgan Hollis
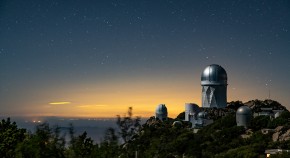
Dark energy is tearing the Universe apart. What if the force is weakening?
The first set of results from a pioneering cosmic-mapping project hints that the repulsive force known as dark energy has changed over 11 billion years, which would alter ideas about how the Universe has evolved and what its future will be.
Quick links
- Explore articles by subject
- Guide to authors
- Editorial policies

Help | Advanced Search
Astrophysics > Cosmology and Nongalactic Astrophysics
Title: the big-bang theory: construction, evolution and status.
Abstract: Over the past century, rooted in the theory of general relativity, cosmology has developed a very successful physical model of the universe: the {\em big-bang model}. Its construction followed different stages to incorporate nuclear processes, the understanding of the matter present in the universe, a description of the early universe and of the large scale structure. This model has been confronted to a variety of observations that allow one to reconstruct its expansion history, its thermal history and the structuration of matter. Hence, what we refer to as the big-bang model today is radically different from what one may have had in mind a century ago. This construction changed our vision of the universe, both on observable scales and for the universe as a whole. It offers in particular physical models for the origins of the atomic nuclei, of matter and of the large scale structure. This text summarizes the main steps of the construction of the model, linking its main predictions to the observations that back them up. It also discusses its weaknesses, the open questions and problems, among which the need for a dark sector including dark matter and dark energy.
Submission history
Access paper:.
- Other Formats
References & Citations
- INSPIRE HEP
- Google Scholar
- Semantic Scholar
BibTeX formatted citation

Bibliographic and Citation Tools
Code, data and media associated with this article, recommenders and search tools.
- Institution
arXivLabs: experimental projects with community collaborators
arXivLabs is a framework that allows collaborators to develop and share new arXiv features directly on our website.
Both individuals and organizations that work with arXivLabs have embraced and accepted our values of openness, community, excellence, and user data privacy. arXiv is committed to these values and only works with partners that adhere to them.
Have an idea for a project that will add value for arXiv's community? Learn more about arXivLabs .

COMMENTS
PDF | Contemporary theories of our Universe, such as the Friedmann-Lemaitre-Robertson-Walker (FLRW) model of the cosmos, assume that time marches on at... | Find, read and cite all the research...
Abstract: I survey physics theories involving parallel universes, which form a natural four-level hierarchy of multiverses allowing progressively greater diversity. Level I: A generic prediction of in ation is an in nite ergodic universe, which contains Hubble volumes realizing all initial conditions.
17 min read. The Evolution of the Universe. Some 15 billion years ago the universe emerged from a hot, dense sea of matter and energy. As the cosmos expanded and cooled, it spawned galaxies,...
An explanation to the present, controversial discussion of expanding accelerating or non-accelerating universe as well as non-expanding universe is given.
Motivated from the above study related to the DP from constant to time dependent this paper aims to investigate the plane symmetric action of the universe within the framework of \(f\left( {R, L_{m} } \right)\) gravity using two different types of DP, constant and time dependent.
Nature Astronomy publishes research, reviews and commentary of the highest quality in all areas of astronomy, astrophysics, planetary sciences.
Cosmology is the study of the universe; its birth, evolution, and ultimate fate. This includes further developing and refining the prevailing model, the Big Bang theory, investigating the...
An elementary survey of mathematical cosmology is presented. We cover certain key ideas and developments in a qualitative way, from the time of the Einstein static universe in 1917 until today.
This publication explores the concept of parallel universes, delving into various theoretical frameworks such as the Many-Worlds Interpretation of quantum mechanics, string theory, and ...
View a PDF of the paper titled The big-bang theory: construction, evolution and status, by Jean-Philippe Uzan. Over the past century, rooted in the theory of general relativity, cosmology has developed a very successful physical model of the universe: the {\em big-bang model}.